Research Overview
Our laboratory research spans the disciplinary boundaries between nanotechnology, biomaterials, and mechanobiology with an emphasis on their applications to tissue engineering and regenerative medicine. Through the use of multi-scale (nano/micro/meso) fabrication and integration tools, we focus on the development and application of bio-inspired materials/devices/systems and functional tissue engineering models for elucidating cell biology, drug screening, disease modeling, and stem cell-based therapies. Using engineered microenvironments in combination with quantitative live cell imaging approaches, we are also studying the intricate interactions between mechanical and biochemical signaling in the regulation of cell/tissue function and fate decisions that are essential for tumor progression and metastasis, tissue repair and regeneration following injury, and various developmental events. The ultimate goal of our research is to better understand complex cellular behavior in response to microenvironmental cues in normal, aging and disease states, to gain new mechanistic insights into the control of cell-tissue structure and function, and to develop multi-scale regenerative technologies for improving human health.
Biologically inspired materials, tissue microenvironment, organs-on-chips, and 3D bioprinting
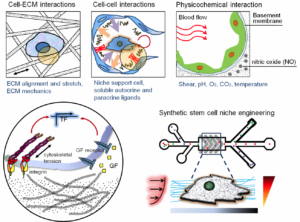
Our laboratory research focuses on engineering combinatorial biomaterial microenvironments through use of variable nano-patterns, and soluble and matrix-bound cell guidance cues in a spatiotemporally controlled manner, which better mimics the in vivo microenvironment under physiological conditions. We develop precisely-controlled biomimetic microenvironments for in vitro cell culture applications through the use of our well-established multi-scale biofabrication techniques, including polymer micro/nano-fabrication, microfluidics, and 3D bioprinting. By more closely mimicking the in vivo microenvironment of specific tissues, in terms of ECM composition, rigidity, and alignment, we can generate synthetic stem cell niche cues better suited to promoting complete maturation of cultured human stem cells which in turn can be employed for more predictive studies of human tissue responses to chemical, mechanical, or pathological challenge. For example, we are developing various types of microfluidic organs-on-chips including a microphysiological 3D human heart on-a-chip assayintegrated with decellularized myocardial matrices to enable the analysis of human pluripotent stem cell-derived cardiomyocyte responses to precise gradients of cardiotoxic compounds. Our decellularized ECM (dECM) has been modified with electroconductive biomaterials (e.g. graphene) to produce electroconductive dECM ‘bioink’ hydrogels with tunable electrical conductivity and stiffness for excitable tissue engineering applications. We utilize these electroconductive dECM bioinks and 3D bioprinting technique to synthesize 3D vascularized whole hearts based on imaging data of native anatomy and vasculature. We are also investigating the potential for using our thermoresponsive nanofabricated substrates (TNFS) to generate scaffold-free 3D cell-dense tissue constructs with precise spatial control of cell-matrix and cell-cell organization for studying the structure-function relationships in complex 3D tissues in healthy and diseased conditions. Within controlled microenvironments enabled by micro- and nanoscale technologies, we strive to systematically characterize the response of live cells to a wide spectrum of dynamically changing mechanical and chemical stimuli. The biomolecular and functional measurements recorded in these studies are highly resolved in time and space, using a variety of live cell probes and well-defined extracellular conditions. For high-throughput quantitative analysis, we are also working to combine a large area nanopatterned substrate with a traditional multi-well tissue culture plate for screening the anti-migratory effect of chemotherapeutic drugs in a high throughput manner. We recently developed an integrated nanogroove-microelectrode array (nanoMEA) device for drug-induced cardiotoxicity screening. We aim to use these tools to gain new mechanistic insights into cell signaling and function, to design new therapies or diagnostic tests for cancer progression and cardiovascular diseases, and to establish organizing principles for development of precisely defined scaffolds for advanced tissue engineering applications.
Systems mechanobiology of cell-cell and cell-matrix interactions
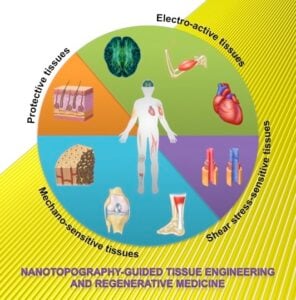
Mechanotransduction – from how cells sense mechanical forces in different tissues to how these mechanical forces are transduced into biochemical signals – is an essential biological process in development, normal physiology and disease. Our research group in this exciting area is particularly interested in investigating the role of mechano-biological processes associated with cell-cell and cell-matrix interactions (e.g. topography, rigidity, dimensionality, etc.) in the regulation of collective cell migration and tissue morphogenesis within controlled microenvironments. Using a combination of various techniques, from live cell imaging analysis to nanotechnology with microrheological measurements, we have been generating interesting data suggesting that one of the most important factors distinguishing malignant from benign cells could be their ability to collectively invade and migrate towards blood vessels by physically interacting with the surrounding extracellular matrices. We combine in-chip live cell culture platforms with time-lapse live cell imaging or FRET (‘fluorescence’ resonance energy transfer) imaging of fluorescently-labeled mechanotransductive signaling proteins (so called “in-chip molecular live cell imaging”). Using these novel tools, our research involves quantitative characterization of the individual and collective behaviors of cancer cells from different stages of the malignant progression using microfabricated tumor tissue models. We particularly focus on analysis of signaling, migration and mechanical properties of highly malignant and invasive tumor cells (vs. benign cells) in glioblastomas, melanomas, and breast cancers. We recently developed a micro/nanofabricated collective migration assay as an enabling tool for analysis and control of cancer cell migration and invasion in a high-throughput, quantitative manner. Using these tools, we also explore the potential role of mechanical guidance in the regulation of tumor progression and invasion under the presence/absence of growth factor-induced signals, and test their biomedical implication by screening cytoskeletal and signal transduction pathways. The proposed high-throughput functional assay for cell migration is a transformative tool for personalized cancer therapy by virtue of its ability to 1) reveal molecular mechanisms of cancer cell invasion relevant to the contexts of specific cancers and 2) provide a functional assay to develop new therapies and ultimately inform patient-specific drug treatments. By experimenting with the nanotopographically-defined cell culture substrates (i.e. quasi 3D cell culture system) and tissue specific dECM bioinks, we are also investigating the role of ECM composition, structure, and mechanics on directed differentiation and functional maturation of cardiomyocytes from human pluripotent stem cells, and the biophysical and signaling mechanisms that underpin these processes. We utilize FRET biosensors, along with systems biology analysis techniques (e.g. mass spectrometry–based proteomics), in order to study the signaling mechanisms that translate exogenous mechanical cues to changes in gene and protein expression. Using this multifaceted approach, we aim to gain a greater understanding of the mechanisms that regulate complex interactions between stem cells and their local microenvironment (or niche), and how manipulation of these pathways can enable the functional maturation of human pluripotent stem cell-derived tissues. These works will provide insight into stem cell and developmental biology and the role of the cardiac microenvironment in controlling cardiac development and maturation in vitro.
Human stem cell and tissue engineering for drug screening, disease modeling, and regenerative therapy
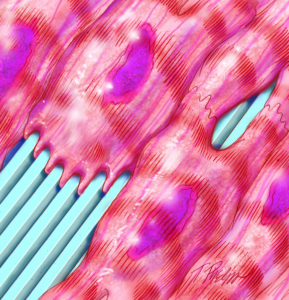
With advances in nanofabrication and biomaterials, scaffolding materials can be designed to integrate biomimetic structural and mechanical cues present in the in vivo ECM environment. Inspired by ultrastructural analyses of the native heart tissue, we utilize our TNFS-based 3D cell sheet engineering technology to develop biomimetic cardiac tissue models to better understand the structure-function relationships in the 3D human heart tissue. We believe that the development of these engineered cardiac tissues will also be applicable in stem cell-based therapies for heart repair and regeneration. The long term goal of this project is to develop functional human cardiac tissue patches for treating damaged heart tissue following myocardial infarction or other cardiomyopathic event. The working hypothesis is that cultivation of cardiac cells on novel biomaterial scaffolds integrated with nanotopographic cues will promote biomimetic anisotropic assembly of uniformly contractile engineered muscle tissue. We further envision that integrating transplantable stem cells with nano-grafting techniques will have therapeutic potential in repairing damaged cardiac tissues, and may help prevent the onset of heart failure. In order to test these hypotheses, our research focuses on elucidating the relationships between scaffold-mediated nanostructural cues and tissue engineered cardiac graft contractility and function. In addition, the therapeutic potential of a nanopatterned cardiac stem cell graft is being studied in vitro and in vivo (implantation onto the infarcted hearts). We are working to characterize structure and function at various hierarchical scales (molecular, single cell, and whole tissue), and the recorded data will be used to tailor the conditions and duration of cultivation, leading to the generation of implantable grafts with correct physiological architectures and functional capacities. Combining our TNFS-based 3D cell sheet engineering technique with a potent angiogenic and myogenic factor such as sphigosine 1-phosphate (S1P), we are also developing 3D vascularized skeletal tissue patches for treatment of diseased skeletal muscles such as Duchenne muscular dystrophy. In order to better understand the development and functional phenotypes of diseased human tissues, we are working to develop disease models of various muscular and neuropathic disease states, including Duchenne Muscular Dystrophy, Familial Cardiomyopathy (MYH7 mutation), and type 2 Charcot Marie Tooth disease (CMT II). Using our multi-scale biofabrication techniques, we are seeking to generate physiologically relevant models of diseased skeletal and cardiac muscle tissues, and neuronal tissues (e.g. neuromuscular junctions) with which to investigate the functional and physiological limitations of such tissues. Through enabling a greater understanding of the development and maturation of diseased tissues, these models will provide valuable information pertaining to new targets for therapeutic treatment. They will also provide meaningful and predictive pre-clinical screens with which to test the efficacy and/or toxicity of new drug compounds, leading to greater clinical success and faster licensing of beneficial new chemical entities.
Funding Sources:
Our research would not be possible without the the generous support of the following public and private organizations.
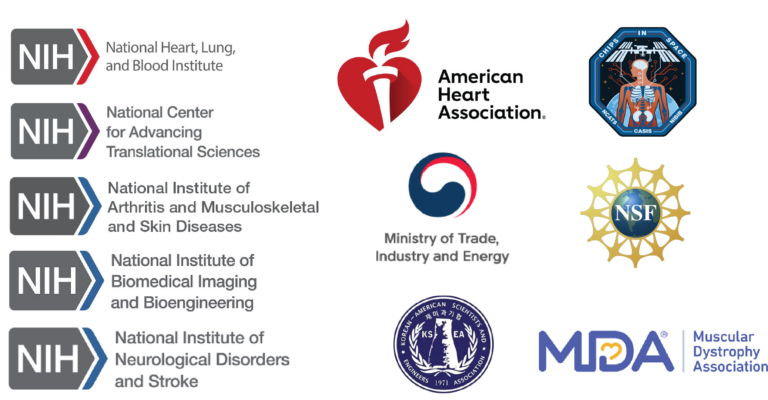